ALTERNATIVE ENERGY SOURCES
The Battery-Electric Flight
The technology for electric drives has been largely optimized and exhausted. Electric motors have an efficiency of up to 97%, that of inverters/controllers is almost 99%. Power connections and battery management systems are also optimized. The sticking point of e-mobility is almost exclusively due to the lack of energy density of batteries.

The media is reporting about energy densities of 400 Wh/kg and more. But where are the actually available energy densities in reality?
Here is an overview of the currently actually achieved battery energy densities at the system level. So not just the bare battery cell, but the actual amount of energy in relation to the total weight of the power supply unit. And only that counts!
Audi e-tron: 95kWh/700kg 136 Wh/kg
Ford Mustang E: 68kWh/485kg 140 Wh/kg
Audi e-tron GT: 93kWh/630kg 148 Wh/kg
Porsche Tycan: 93kWh/630kg 148 Wh/kg
Jaguar I-pace: 90kWh/599kg 150 Wh/kg
BYD Han EV: 85kWh/568kg 150 Wh/kg
Mercedes EQS: 108 kWh/692kg 156 Wh/kg
Tesla S: 100kWh/625kg 160 Wh/kg
Tesla 3: 75kWh/439kg 171 Wh/kg
Tesla Y: 75kWh/437kg 172 Wh/kg
Rimac: 120kWh/599kg 200 Wh/kg
The average brutto energy density is therefore 157 Wh/kg. And these energy densities are also given without the weight of cooling liquid, pipes, pumps, coolers, etc. The usable energy is normally approx. 5 - 10% less.
Taking all these factors into account, the average actual realized net energy densities that can actually be used for propulsion is below 150 Wh/kg.
The significantly higher requirements of aviation apply to aircraft batteries. In particular, crash safety and fire protection play a much greater role than with cars. Because in the event of a fire it is not possible to stop and exit the aircraft. This further reduces the actually available energy density.
​
For example the Pipistrel aviation battery: PB345V124E-L
A 10.35 kWh unit weighs 74 kg = 140 Wh/kg energy density. Even that is calculated without cables, hoses, coolant, pump, cooler, crash-proof housing, etc. More information can be found HERE.
Airplanes are about 10 times more weight sensitive than cars. Even if the battery-electric drive for road traffic is emerging as the future solution, this is much more difficult for the significantly weight-sensitive aircraft.
The following physical energy consideration is intended to show what performance is currently achievable for cruising with battery-powered aircraft.
Battery (2x Pipistrel PB345V124E-L) 20kWh
Losses (motor, inverter, cable, ...) -10%
Available drive energy 18kWh
Acceleration of the aircraft and climb to
cruising altitude -1.2kWh
legal minimum reserve 30 min. (20 kW / 27 hp) - 10kWh
remaining energy for planned cruise flight 6.8kWh
What flight time can be achieved with 6.8 kWh of energy ?
standard minimum
Assumed cruising power 70 hp (51,5kW) 41 hp (30kW)
legally plannable cruise time 8,2 min. 13,6 min.
This calculation does not take into account the power consumption for the avionics, electric landing flaps, retractable landing gear or even a cockpit heating!
It can therefore be assumed that purely battery-powered sport aircraft will remain largely restricted to operation near airports, at least for the next 25-30 years, unless a battery miracle occurs.
Range
Even efficient aircraft can hardly be sold with tanks of less than 70 liters. This achieves a minimum flight time of 4 hours and a range of at least 800 km with a power of around 70 hp (51.5 kW).
The vast majority of general aviation pilots see this as the absolute minimum requirement when purchasing an aircraft.
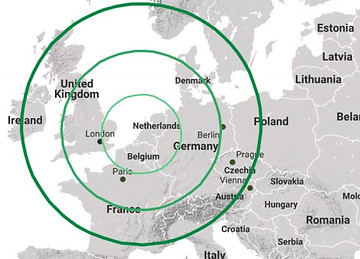
Here's a look at the weight calculation:
As stated above, a cruising performance of 70 hp / 51.5 kW should be available over a period of 4 hours.
​
Assuming an energy density of 200 Wh/kg, a battery would weigh around 1.145 kg with today's most modern battery technology (51.5 kW / 90% efficiency x 4 hours = 229 kWh / 200 Wh/kg = 1145 kg).
For comparison:
A full 70-litre gasoline tank including the pump, hoses, etc. weighs around 58 kg, with which a light aircraft with a take-off weight of 600 kg can be operated for 4 hours with 70 hp!
(51.5 kW x 0.25 kg/kWh (specific consumption) x 4 hours / 0.73 (specific weight of gasoline) = 70 liters)
This results in the well-known weight factor of 1 : 20 (1145/58 kg)
​
This means that a battery system is around twenty times heavier than a fuel system in order to bring the same energy to the propeller. And that even with the best and new batteries currently available.
​
But as we know, every kilogram counts in aviation!
This clearly shows that there is a long way from replacing piston engines in airplanes with battery electric drives. Only a fraction of the demand can be met with batteries or hydrogen for the next 25-30 years or more.
​
So, we need a future-oriented technology for the next 30 years and probably longer - XAEROS is developing it!
Hydrogen as Energy Source
Hydrogen does not occur in nature in a directly usable form. Hydrogen has to be technically produced in order to make it usable. Hydrogen is always colorless, but several names have become common to distinguish it in terms of the manufacturing process.
The so-called green hydrogen is produced by electrolysis. Here, the so-called renewable energy is obtained from water, sun and wind power for the splitting of water (H2O) into hydrogen and oxygen. This process is only possible with an efficiency of around 80%. This means that 20% of the valuable renewable energy is already lost in the first step.
​
We will urgently need the valuable regenerative energies for battery electric vehicles in road traffic and many other applications for which electrical energy can hardly be replaced. The switch from gas heating to heat pumps and the energy transition in industry require additional enormous amounts of renewable energy. It can therefore not be assumed that in temperate climate zones so much solar and wind energy can ever be generated that green hydrogen can also be produced locally for mobility.
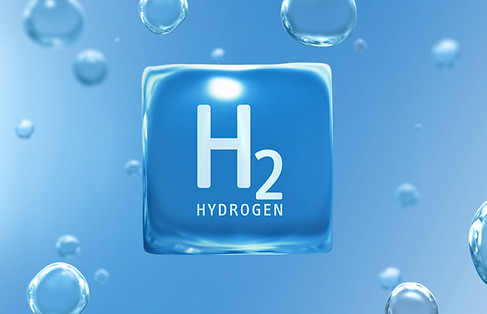

Hydrogen would therefore have to be produced for the most part in sunny and windy regions around the world. However, hydrogen is not so easy to transport across the oceans in large tankers. This requires tankers with very well insulated pressure vessels that can transport the hydrogen in liquefied form, i.e. cooled down to minus 253.
The latest and largest hydrogen tanker model was developed by Dutch energy company LH2 Europe. It will not be operational until 2027.
The 142 meter long liquid hydrogen tanker holds 37,500 m³. However, liquefied hydrogen is also very light at only 71 kg/m³, which means that this largest pressure tank tanker can only transport 2,660 tons of liquefied hydrogen. A proportion of around 15 - 20% of this is also lost through boil-off while driving.
This means that only around 2,260 tons of hydrogen arrive at the port of destination, which corresponds to energy of around 75 million kWh.
In comparison, a medium-sized oil tanker, which can also be used to transport eFuels, holds around 250,000 tons, which corresponds to an energy of 3 billion kWh.
So, 40 hydrogen tankers can transport as much energy as one tanker for eFuels!
Conversion of hydrogen to ammonia to achieve higher energy density for transport is also being considered. However, around 45% of the energy is lost in the conversion process (Haber-Bosch). Another 10% of the energy is lost when converting ammonia back into hydrogen.
Although ammonia in liquid form is almost 10 times heavier than hydrogen, but ammonia has only 15% of the energy density of hydrogen.
To transport the same amount of energy as with existing eFuel tankers, you therefore need 27 ammonia tankers, which would first have to be built.
In addition, oil, diesel and kerosene tankers are gradually becoming free as a result of the energy transition and can be used to transport eFuels. Hydrogen tankers do not currently exist and would have to be developed, produced and deployed from scratch. Huge investments would be required here.
Other manufacturing processes, such as so called "gray hydrogen", use natural gas, i.e. methane, as the basis. When converting methane into hydrogen, which is how most of the hydrogen produced today is produced, around 10 kg of CO2 are released per kg of hydrogen, which is why this is very questionable from an environmental point of view.
​
Hydrogen as an energy carrier is associated with difficult-to-solve challenges in General Aviation:
Infrastructure
-
the majority of general aviation aircraft are flown at small airports, very often in club operations. It is unlikely that airports and clubs would invest around €1 million per hydrogen filling station if they can buy 3-6 brand new aircraft for it. The question also arises as to whether investing in a hydrogen filling station will ever pay off.
Technology ​
-
enormous weight and extensive technology of the components (tanks, fuel cell, lines, technology, etc.)
-
large volume of hydrogen tanks
-
Converting existing aircraft is very difficult. This means that new aircraft for hydrogen propulsion would have to be developed, produced and purchased.
It can be assumed that it would therefore take decades before the switch to hydrogen-powered aircraft would have any impact on environmental protection, which urgently needs to be promoted.
Synthetic Fuels - eFuel/SAF (sustainable aviation fuel)

Synthetic fuels are made from CO2 from the air, water and solar or wind energy. Exactly as much CO2 is absorbed from the air during production as is later released again during combustion. Therefore, with synthetic fuels, so-called eFuels, a CO2-neutral operation of aircraft is possible.
​
The efficiency in the production of eFuels is lower than in the production of hydrogen, since hydrogen is the starting point for the production of eFuels. However, liquid fuels have the advantage that transport is much easier and cheaper than that of hydrogen and the infrastructure required for transport to refueling at the airport is already available. This means that the transport of eFuels over long distances is cost-effective and much more energy-efficient.
​
Synthetic fuels can therefore be produced where there is an abundance of regenerative energy, i.e. in sunny and windy regions around the world. With the same investment, installing a solar system in a desert region produces around three times the amount of green energy compared to systems in central Europe, for example. This more than compensates for the non-optimal efficiency in the production of eFuels. Transport ships can also be operated CO2-neutrally with eFuels.
CONCLUSION
Developing new aircraft for battery-electric or hydrogen-based propulsion would take many decades. It would take another decade before the new aircraft are actually in operation and the first noticeable effects in terms of CO2-reducing operation become apparent. Quite independently of this, the question arises as to whether the required ranges and requirements of aviation can ever be achieved with these technologies.
eFuels do not make sense where battery electric solutions are applicable. With eFuels, however, air and ship traffic can be converted to CO2-neutral operation in the foreseeable future. This is possible with the existing ship and aircraft fleets. The entire infrastructure network is also available for this. As a result, appropriate measures can be taken with regard to the urgently needed environmental protection in a much shorter period of time.
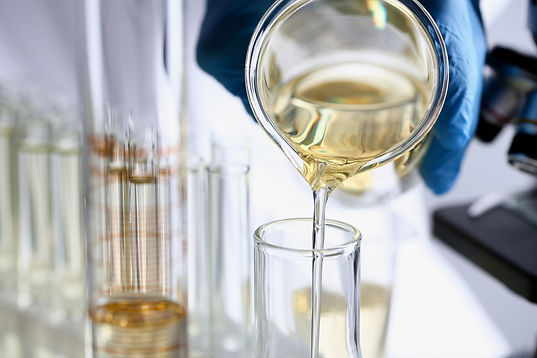